Earthquakes, also known as seismic events, are powerful natural phenomena that can dramatically alter the Earth’s surface in mere moments. These sudden and intense tremors are caused by the release of energy accumulated due to the movement of tectonic plates—large, rigid slabs of the Earth’s lithosphere that float on the more fluid asthenosphere beneath them. As these tectonic plates interact at their boundaries—whether converging, diverging, or sliding past each other—stress builds up until it exceeds the strength of the rocks, causing them to fracture and slip. This release of energy generates seismic waves that propagate outward, resulting in the shaking of the ground and, in severe cases, leading to substantial damage and loss of life.
Understanding the fundamental mechanics of earthquakes is vital for several reasons. Knowledge of how seismic waves are generated and how they travel through the Earth helps in designing earthquake-resistant structures and developing effective preparedness plans. Studying earthquakes also plays a crucial role in assessing risk, implementing mitigation strategies, and advancing technological innovations aimed at improving our ability to predict and respond to these events. By delving into the science of earthquakes, we enhance our capability to safeguard communities, minimize damage, and improve resilience in the face of these powerful natural occurrences.
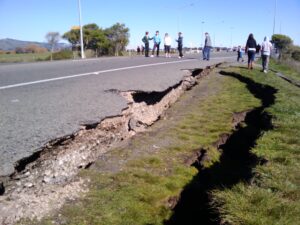
(flickr.com)
Earthquake Description
An earthquake, also known as a seismic event, is a sudden and intense shaking or trembling of the Earth’s surface caused by the release of energy from the Earth’s crust. This release of energy results from the movement of tectonic plates—large, rigid slabs of the Earth’s lithosphere that slowly drift atop the more fluid asthenosphere beneath them. These tectonic plates interact at their boundaries, where they may converge, diverge, or slide past each other. The friction and stress that accumulate at these plate boundaries or within the plates themselves eventually become too great, causing the rocks to fracture and slip. This sudden movement generates seismic waves that propagate outward, causing the ground to shake and, in some cases, leading to significant structural damage and loss of life.
The basic mechanics of an earthquake involve several key components:
Fault Lines: Faults are fractures in the Earth’s crust where blocks of rock have moved relative to each other. Most earthquakes occur along these faults, which can range from small, localized fractures to large, continental-scale boundaries.
Focus (Hypocenter): The focus is the point within the Earth where the earthquake originates. It is the initial point of the rupture that produces the seismic waves.
Epicenter: The epicenter is the point on the Earth’s surface directly above the focus. It is often the location where the shaking is felt most intensely and where damage is usually most severe.
Seismic Waves: Seismic waves are the energy waves that travel through the Earth’s crust and include:
- Primary Waves (P-waves): These are compressional waves that travel the fastest and are the first to be detected by seismographs. They move through solids, liquids, and gases.
- Secondary Waves (S-waves): These waves are shear waves that follow the P-waves. They only move through solids and are typically more destructive than P-waves.
- Surface Waves: These waves travel along the Earth’s surface and are responsible for most of the shaking experienced during an earthquake.
Studying earthquakes is crucial for several reasons:
Safety and Preparedness: Understanding earthquake mechanics helps in designing buildings and infrastructure that can withstand seismic activity. It also aids in creating effective emergency preparedness plans, including evacuation strategies, and public education about how to respond before, during, and after an earthquake.
Risk Assessment and Mitigation: Earthquake research allows scientists to assess the seismic risk of various regions, leading to better land-use planning and construction practices. By identifying high-risk areas, communities can implement mitigation strategies to reduce potential damage and losses.
Technological Advancements: Ongoing research into earthquakes has led to significant technological advancements, such as seismic monitoring networks, early warning systems, and improved building materials. These innovations can enhance our ability to predict and respond to seismic events, potentially saving lives and reducing economic impacts.
Understanding the science of earthquakes, improving preparedness measures, and investing in technological advancements are essential for minimizing the impact of these natural events and safeguarding communities. By continuing to study and learn about earthquakes, we can enhance our resilience and adaptability in the face of seismic challenges.
The Science of Earthquakes
(Tectonic Plates and Fault Lines)
Earthquakes are primarily caused by the movement of tectonic plates, which are large, rigid slabs of the Earth’s lithosphere floating atop the semi-fluid asthenosphere beneath them. The Earth’s crust is divided into several major and minor tectonic plates that are in constant motion due to convective currents in the mantle. These interactions occur at plate boundaries, which can be classified into three main types. At convergent boundaries, two plates collide or move toward each other, often resulting in one plate being forced beneath another. This process creates intense pressure and stress, which can be released suddenly, causing an earthquake. The release of this built-up stress at convergent boundaries is a primary source of seismic activity.
Divergent boundaries, in contrast, involve plates moving away from each other, creating a gap that is filled by rising magma. This magma solidifies to form new crust as the plates separate. The tension and stretching at divergent boundaries can also lead to earthquakes. As the plates move apart, the gaps created can cause stress to accumulate in the surrounding rock, which, when released, results in an earthquake. This type of seismic activity is commonly observed along mid-ocean ridges and rift valleys where new crust is constantly being generated.
Finally, transform boundaries are characterized by plates sliding past each other horizontally. The friction between the sliding plates can cause them to become locked together, preventing movement until the accumulated stress exceeds the frictional force. When this stress is finally released, it results in an earthquake. Fault lines, which are fractures in the Earth’s crust where this movement occurs, are categorized based on their movement direction: normal faults, where the hanging wall drops relative to the footwall; reverse faults, where the hanging wall rises relative to the footwall; and strike-slip faults, where rocks slide past each other horizontally. Each type of fault contributes to the overall seismic activity experienced during an earthquake.
Seismic waves are energy waves generated by the sudden release of stress during an earthquake. These waves propagate through the Earth’s crust and are responsible for the shaking experienced during seismic events. They are categorized into two main types: body waves and surface waves. Body waves travel through the Earth’s interior and are further subdivided into Primary Waves (P-waves) and Secondary Waves (S-waves). P-waves are compressional waves that move in a push-pull motion, allowing them to travel through solids, liquids, and gases. As the fastest seismic waves, P-waves are the first to be detected by seismographs, providing early warning of an impending earthquake.
In contrast, S-waves, or Secondary Waves, are shear waves that move perpendicular to the direction of wave propagation. They travel only through solids and are typically slower than P-waves. S-waves cause the ground to move side-to-side or up-and-down, which often results in more intense shaking and greater structural damage. The movement of S-waves contributes significantly to the destructive impact of an earthquake, as they generate more violent ground motion compared to P-waves.
Surface waves, which travel along the Earth’s surface, generally cause the most damage during an earthquake. They include Love Waves and Rayleigh Waves. Love Waves cause horizontal shearing of the ground, leading to side-to-side motion that can severely affect buildings and infrastructure. Rayleigh Waves produce an elliptical rolling motion that resembles ocean waves, moving both vertically and horizontally. This rolling motion can cause significant ground deformation and structural damage. The combined effects of surface waves often result in the most noticeable and destructive shaking experienced during seismic events.
(Magnitude and Intensity)
Magnitude and intensity are two essential measurements used to describe earthquakes, each addressing different aspects of a seismic event. Magnitude quantifies the amount of energy released at the earthquake’s source and is determined through seismographic data. The Richter Scale, developed in 1935, was one of the earliest methods for measuring magnitude. It assesses the amplitude of seismic waves recorded by seismographs. The scale is logarithmic, which means each whole number increase represents a tenfold increase in amplitude and approximately 31.6 times more energy released. While the Richter Scale is still used, it is less effective for very large earthquakes or those occurring at great distances from the seismograph.
To address these limitations, the Moment Magnitude Scale (Mw) was developed as a more accurate measure for large earthquakes. This scale considers factors such as the size of the fault, the amount of slip along the fault, and the properties of the rocks involved in the rupture. By providing a comprehensive measure of the total energy released, the Moment Magnitude Scale offers a better understanding of the earthquake’s overall impact. It is now the preferred scale for quantifying the magnitude of significant seismic events and providing a consistent measure across various distances and earthquake sizes.
In contrast, intensity measures the effects of an earthquake at specific locations, such as the extent of damage and the level of shaking experienced. This qualitative measure is assessed using scales like the Modified Mercalli Intensity (MMI) Scale, which ranges from I (not felt) to XII (total destruction). The MMI Scale categorizes intensity based on observed effects, including structural damage, changes in the landscape, and human reactions. Understanding both magnitude and intensity is crucial for assessing the overall impact of an earthquake, as magnitude provides insight into the energy released, while intensity reveals the earthquake’s effects on people and infrastructure. This comprehensive understanding informs emergency response and preparedness efforts, helping communities better prepare for and recover from seismic events.
Historical Earthquakes and Their Impact
Great Earthquake of Lisbon (1755)
The Great Earthquake of Lisbon, which struck on November 1, 1755, was one of the most devastating seismic events in European history. The earthquake, which registered an estimated magnitude of 8.5 to 9.0, struck Lisbon, Portugal, during the Feast of All Saints, leading to a catastrophic loss of life and widespread destruction. The quake caused massive destruction in Lisbon, collapsing buildings, churches, and even the city’s fortifications. The devastation was compounded by a series of aftershocks and a subsequent tsunami, which inundated the Portuguese coastline, further exacerbating the damage and loss of life.
The impact of the earthquake was profound, not only on the city of Lisbon but also on European society and architecture. The disaster prompted a reevaluation of architectural practices and urban planning. In the wake of the earthquake, the Portuguese Prime Minister, Sebastião José de Carvalho e Melo (the Marquis of Pombal), spearheaded a major reconstruction effort. The rebuilding of Lisbon introduced new standards for earthquake-resistant construction, including the use of reinforced stone and the creation of wide, straight streets to facilitate better evacuation and firefighting.
The earthquake also had a significant influence on European thought, affecting the fields of philosophy, economics, and seismology. It led to the development of the modern science of seismology and raised questions about divine providence and the role of natural disasters in human affairs.
San Francisco Earthquake (1906)
The San Francisco Earthquake of April 18, 1906, was a major seismic event that dramatically reshaped the city of San Francisco and had a lasting impact on earthquake engineering and urban planning. With an estimated magnitude of 7.8, the earthquake caused widespread destruction across San Francisco and the surrounding areas. The quake and the subsequent fires that ignited due to broken gas lines destroyed approximately 80% of the city, leaving around 250,000 people homeless and resulting in an estimated 3,000 deaths. The devastation highlighted the vulnerabilities in building construction and urban infrastructure.
In the aftermath of the earthquake, significant changes were implemented in building codes and urban planning. The disaster prompted a rethinking of construction practices, leading to the adoption of stricter building codes designed to enhance structural resilience against seismic events. The principles of earthquake engineering, including the importance of flexible building materials and the need for rigorous safety standards, became more widely recognized and integrated into building regulations.
Additionally, the reconstruction efforts in San Francisco emphasized the development of improved urban planning practices, including better infrastructure and emergency response systems. The lessons learned from the 1906 earthquake have influenced earthquake preparedness and building standards not only in California but also globally.
Tohoku Earthquake (2011)
The Tohoku Earthquake, which struck Japan on March 11, 2011, was one of the most powerful earthquakes ever recorded, with a magnitude of 9.0. The quake, centered off the coast of the Tohoku region, triggered a massive tsunami that devastated coastal areas and led to the Fukushima Daiichi nuclear disaster. The earthquake and tsunami caused extensive damage to infrastructure, homes, and communities, resulting in nearly 16,000 deaths and significant economic losses.
The earthquake’s impact extended beyond the immediate destruction, particularly through the Fukushima nuclear disaster. The tsunami overwhelmed the cooling systems at the Fukushima Daiichi Nuclear Power Plant, leading to core meltdowns, radiation releases, and the evacuation of tens of thousands of residents. The disaster highlighted vulnerabilities in nuclear safety and led to a global reassessment of nuclear energy policies and practices.
In Japan, the event spurred changes in nuclear regulations, disaster preparedness, and infrastructure resilience. Internationally, the Tohoku Earthquake underscored the need for improved global cooperation in disaster response and risk management. The lessons learned from the Tohoku Earthquake continue to influence disaster preparedness and response strategies worldwide, emphasizing the importance of robust infrastructure and comprehensive emergency planning to mitigate the impact of future seismic events.
Earthquake Preparedness and Safety Measures
(Building Design and Engineering)
Modern building design and engineering have made significant strides in earthquake preparedness to mitigate the impact of seismic activity. One key innovation is the use of base isolators, which are designed to absorb and dissipate seismic energy. Base isolators are placed between a building’s foundation and superstructure, allowing the building to move independently of the ground motion. This helps to reduce the amount of shaking transferred to the building, thereby minimizing structural damage.
Flexible structures are another crucial advancement in earthquake-resistant design. These buildings are engineered to bend and sway without collapsing, thanks to materials and construction techniques that allow for controlled movement. For example, modern skyscrapers often incorporate damping systems that absorb and dissipate seismic energy, such as tuned mass dampers or viscous dampers. These systems help to stabilize buildings during an earthquake, reducing the potential for damage and improving safety.
Additionally, retrofitting existing buildings to enhance their seismic resilience is a common practice. This involves strengthening structures by adding reinforcements, such as steel braces or concrete shear walls, to improve their ability to withstand earthquake forces. Advances in engineering and materials science continue to drive innovations in building design, making new and existing structures more resilient to seismic events.
(Emergency Preparedness)
Effective earthquake preparedness is crucial for minimizing harm and ensuring a swift recovery. Individuals and communities should follow several guidelines to prepare for seismic events. Creating an emergency kit is a fundamental step; it should include essential items such as water, non-perishable food, a flashlight, batteries, a first-aid kit, and any necessary medications. It’s also important to have a communication plan that includes contact information for family members and neighbors, as well as a designated meeting place in case of evacuation.
Developing an evacuation plan is another critical aspect of earthquake preparedness. This plan should outline safe exit routes from homes, workplaces, and schools, and include procedures for quickly evacuating if necessary. Practicing earthquake drills regularly helps individuals and families become familiar with safety procedures and ensures a more organized response during an actual event. Communities should also engage in public education initiatives to raise awareness about earthquake risks and preparedness measures.
(Government and Institutional Roles)
Governments and institutions play a pivotal role in earthquake preparedness and response. Early warning systems are one of the key tools used to detect and alert communities about impending earthquakes. These systems use a network of seismographs and sensors to detect seismic activity and provide advance notice to areas that might be affected, allowing people to take protective actions before the shaking begins.
Public education campaigns are essential for informing residents about earthquake risks and safety measures. Governments and institutions often collaborate on initiatives to educate the public about earthquake preparedness, proper building practices, and emergency response strategies. Additionally, regulatory agencies enforce building codes and standards that ensure new and retrofitted structures are designed to withstand seismic forces.
In the aftermath of an earthquake, governments and organizations coordinate disaster response efforts, including rescue operations, medical aid, and infrastructure repairs. International organizations also play a role in providing support and resources to affected areas. By working together, governments, institutions, and communities can improve resilience and effectively manage the challenges posed by seismic events.
Advances in Seismology and Earthquake Prediction
(Seismic Monitoring Technology)
Seismic monitoring technology has advanced significantly, providing crucial tools for detecting and analyzing seismic activity. Seismometers, also known as seismographs, are the primary instruments used to record seismic waves. These devices measure ground motion by detecting vibrations and movements caused by earthquakes. Modern seismometers are highly sensitive and can detect even the smallest seismic signals, providing valuable data for analyzing earthquake magnitude, location, and depth.
In addition to seismometers, Global Positioning System (GPS) technology plays a critical role in monitoring seismic activity. GPS systems measure the precise movements of tectonic plates by tracking changes in the Earth’s surface. By analyzing data from a network of GPS stations, scientists can observe how tectonic plates shift and deform over time. This information helps in understanding the buildup of stress along fault lines and provides insights into potential earthquake risks. Combining GPS data with seismic measurements allows for a more comprehensive view of tectonic activity and improves our ability to assess earthquake hazards.
InSAR (Interferometric Synthetic Aperture Radar) is another advanced technology used in seismic monitoring. InSAR uses satellite radar to measure ground deformation with high precision. By comparing radar images taken before and after seismic events, scientists can detect changes in the Earth’s surface, such as uplift or subsidence. This data is crucial for understanding the impact of earthquakes and for identifying areas of potential future activity.
(Earthquake Prediction Challenges)
Predicting earthquakes with precision remains one of the most challenging aspects of seismology. Despite significant advances in monitoring technology, accurately forecasting the time, location, and magnitude of an earthquake is still elusive. One major challenge is the complexity of the Earth’s crust and the variety of factors that influence seismic activity. Earthquakes result from the accumulation and release of stress along faults, but predicting when and where this stress will lead to a rupture is difficult.
Another challenge is the lack of consistent precursors or warning signs that reliably indicate an impending earthquake. While some potential indicators, such as foreshocks or changes in groundwater levels, have been observed, they are not always present or easy to interpret. Additionally, the behavior of faults and the distribution of stress are highly variable, making it difficult to develop a one-size-fits-all prediction model.
Statistical and probabilistic approaches are used to estimate the likelihood of earthquakes over a given period and region, but these methods provide only general probabilities rather than specific predictions. Researchers continue to study earthquake patterns and seek better understanding of the conditions leading up to seismic events, but the inherent complexity of the Earth’s geology makes precise prediction a formidable challenge.
(Recent Innovations)
Recent research and advancements in earthquake prediction and mitigation technologies hold promise for improving our understanding and preparedness. One significant innovation is the development of early warning systems that provide alerts seconds to minutes before shaking begins. These systems use data from seismometers to detect the initial, less-damaging P-waves of an earthquake and issue warnings to affected areas, allowing people to take protective actions.
Machine learning and artificial intelligence are also being explored to enhance earthquake prediction capabilities. These technologies analyze vast amounts of seismic data to identify patterns and potential precursors that might indicate an imminent earthquake. Machine learning algorithms can process and interpret complex data sets more quickly than traditional methods, potentially improving forecasting accuracy.
Advancements in seismic hazard modeling and risk assessment are helping to refine our understanding of earthquake impacts. Researchers use detailed simulations and models to predict how seismic waves will travel through different types of soil and rock, allowing for better assessment of potential damage. These models inform building codes and disaster preparedness plans, contributing to more resilient infrastructure and improved community readiness.
Overall, while precise earthquake prediction remains a challenge, ongoing research and technological innovations are enhancing our ability to monitor seismic activity, understand earthquake processes, and mitigate their impacts. Continued investment in these areas is crucial for improving safety and resilience in earthquake-prone regions.
Case Studies: Innovations and Success Stories
(Japan’s Earthquake-Resistant Infrastructure)
Japan is renowned for its advanced earthquake-resistant infrastructure and preparedness measures, which have significantly minimized damage during recent seismic events. The country’s approach to earthquake engineering is a result of its long history of frequent seismic activity, leading to the development of innovative technologies and building practices designed to enhance resilience.
One of the key features of Japan’s earthquake-resistant infrastructure is the use of base isolators and damping systems. Base isolators are placed between a building’s foundation and its structure, allowing the building to move independently of ground motion. This technology absorbs and dissipates seismic energy, reducing the impact of earthquakes. Damping systems, such as tuned mass dampers, are integrated into high-rise buildings to counteract swaying caused by seismic activity. These systems help stabilize structures and minimize potential damage.
Japan also employs rigid structural frameworks and flexible materials in construction to ensure that buildings can withstand seismic forces. The country’s building codes are among the strictest in the world, requiring rigorous testing and adherence to seismic standards. Additionally, Japan has invested in early warning systems that provide alerts seconds to minutes before shaking begins. These systems use data from seismometers and GPS to detect the initial P-waves of an earthquake and issue warnings to residents and infrastructure operators, allowing for timely responses and protective measures.
The combination of advanced engineering, stringent building codes, and early warning systems has contributed to Japan’s ability to minimize damage and casualties during earthquakes. For example, during the 2011 Tohoku Earthquake, despite the magnitude of the event and the resulting tsunami, the earthquake-resistant infrastructure and preparedness measures helped reduce the overall impact on many buildings and critical infrastructure.
(Community Resilience in Chile)
Chile, another earthquake-prone country, has demonstrated remarkable community resilience and preparedness in the face of frequent seismic events. The country’s experience with major earthquakes, including the devastating 2010 Maule Earthquake, has led to significant efforts in rebuilding and preparing for future seismic events.
Following the 2010 Maule Earthquake, which registered a magnitude of 8.8, Chile embarked on an extensive rebuilding effort. The reconstruction process emphasized seismic-resistant construction and the reinforcement of existing buildings. New building codes were implemented, requiring structures to meet stringent earthquake-resistant standards. These codes include provisions for ductile materials, which can bend without breaking, and reinforced concrete, which enhances the strength of buildings.
Local communities played a crucial role in the recovery process, with grassroots organizations and community-based initiatives driving efforts to rebuild and prepare for future earthquakes. These efforts included community workshops and educational programs to raise awareness about earthquake preparedness and safety. The involvement of local residents in the planning and rebuilding process helped ensure that the measures implemented were effective and tailored to the specific needs of the community.
Chile’s commitment to disaster preparedness and response has also led to the development of comprehensive emergency plans and evacuation strategies. These plans involve regular drills, public education campaigns, and coordination with national and local authorities. The focus on community resilience and preparedness has contributed to a more robust response to seismic events and has helped mitigate the impact of subsequent earthquakes.
The success of these initiatives in Chile is evident in the country’s ability to recover and rebuild after seismic events. The emphasis on community involvement, coupled with improved building practices and preparedness measures, has strengthened Chile’s resilience to earthquakes and has provided valuable lessons for other earthquake-prone regions around the world.
Overall, both Japan and Chile provide inspiring examples of how innovative engineering, stringent building codes, and community resilience can significantly enhance earthquake preparedness and minimize the impact of seismic events. These case studies highlight the importance of proactive measures and collaborative efforts in building safer and more resilient communities.
The Future of Earthquake Science and Safety
(Ongoing Research)
The future of earthquake science is driven by ongoing research aimed at enhancing our understanding of seismic events and improving prediction capabilities. Current research efforts focus on several key areas:
Seismic Hazard Assessment: Researchers are working on advanced seismic hazard models to better understand and predict the potential impact of earthquakes. These models incorporate data on fault lines, tectonic plate movements, and ground conditions to assess the likelihood of earthquakes and their potential effects on specific regions. Enhanced hazard models help in designing more resilient infrastructure and informing land-use planning.
Earthquake Precursors and Indicators: Scientists are investigating potential earthquake precursors, such as changes in groundwater levels, gas emissions, and electromagnetic anomalies. Identifying reliable precursors could improve short-term prediction capabilities. Research is also focused on understanding the behavior of faults and the conditions that lead to seismic rupture, which could help in developing more accurate forecasting methods.
Early Warning Systems: Advances in early warning systems are a critical area of research. Scientists are exploring ways to improve the accuracy and speed of detection, as well as expanding the reach of these systems to cover more regions. Research includes enhancing the algorithms used to process seismic data and integrating multiple data sources, such as satellite observations and real-time ground motion measurements.
Seismic Resilience Technologies: Research is ongoing into new technologies and materials that can improve earthquake resilience in buildings and infrastructure. This includes the development of advanced damping systems, smart materials that can adapt to seismic forces, and innovative construction techniques that enhance structural stability.
Community-Based Research: There is a growing emphasis on community-based research to understand how local knowledge and practices can complement scientific efforts. Studies focus on improving public education, emergency preparedness, and community engagement in disaster planning and response.
(Global Collaboration)
The future of earthquake science and safety also hinges on international cooperation and collaboration. The importance of global collaboration in this field cannot be overstated, as earthquakes are a global phenomenon that requires a collective effort to address effectively:
Sharing Knowledge and Data: International collaboration facilitates the sharing of seismic data, research findings, and best practices. Organizations such as the International Seismological Centre and the Global Earthquake Model (GEM) Foundation provide platforms for the exchange of information and the development of global seismic hazard models. By pooling resources and expertise, scientists can gain a more comprehensive understanding of earthquake processes and improve prediction and preparedness efforts.
Collaborative Research Initiatives: Global research initiatives bring together scientists, engineers, and policymakers from different countries to work on common goals. Programs like the United Nations Office for Disaster Risk Reduction (UNDRR) and the International Organization for Standardization (ISO) work on developing international standards for earthquake-resistant construction and disaster preparedness. Collaborative research helps in addressing knowledge gaps and implementing effective solutions on a global scale.
Disaster Response and Recovery: International cooperation is crucial during disaster response and recovery efforts. Countries affected by earthquakes often receive assistance from international organizations and neighboring nations, including humanitarian aid, technical support, and expertise in recovery and reconstruction. The global network of disaster relief organizations, such as the Red Cross and Doctors Without Borders, plays a vital role in providing timely assistance and coordinating recovery efforts.
Education and Training: Global collaboration extends to education and training programs aimed at building capacity and raising awareness about earthquake safety. International conferences, workshops, and training sessions provide opportunities for knowledge exchange and skill development. Programs such as the International Disaster Emergency Service (IDES) offer training for emergency responders and public officials in earthquake-prone regions.
Policy Development: Collaborative efforts in policy development help in creating and implementing effective earthquake preparedness and mitigation strategies. International bodies, such as the International Federation of Red Cross and Red Crescent Societies (IFRC), work with governments to develop policies that enhance resilience and ensure that resources are allocated efficiently.
Overall, the future of earthquake science and safety relies on the continued advancement of research and the strengthening of global partnerships. By working together, scientists, engineers, policymakers, and communities can improve our ability to predict, prepare for, and respond to seismic events, ultimately reducing the impact of earthquakes and enhancing resilience worldwide.
Conclusion,
In conclusion, understanding earthquakes—their causes, mechanics, and impacts—is crucial for enhancing our preparedness and resilience against these powerful natural events. By studying the movement of tectonic plates, the generation of seismic waves, and the resultant shaking of the ground, we gain valuable insights that help in designing earthquake-resistant structures and developing effective emergency response plans. The knowledge gained from earthquake research not only aids in risk assessment and mitigation but also drives technological advancements that improve our ability to predict and respond to seismic activities.
Global collaboration and continued research efforts are essential in advancing earthquake science and safety. Sharing data, resources, and expertise on an international scale allows for a comprehensive approach to understanding and managing earthquake risks. As we continue to innovate and implement new strategies, we can better protect communities, reduce the potential for damage, and ultimately save lives. By fostering a proactive and informed approach to earthquake preparedness, we enhance our capacity to adapt and thrive in the face of seismic challenges, ensuring a safer and more resilient future for all.